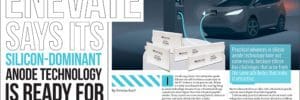
Q&A with Enevate’s Founder and CTO Dr. Benjamin Park
Introducing silicon into automotive-grade lithium-ion cells has been a major topic in the EV industry in the past decade. Silicon is widely considered to be the next big thing in anode technology, because it has a theoretical charge capacity ten times higher than that of typical graphite anodes. Many experts see a race among battery makers to get more and more silicon into their anodes.
Replacing the graphite in a cell with silicon means that you can use less anode material, and fill up the extra space with more cathode material—effectively increasing the overall energy that can be stored within the same volume. This is due to a fundamental difference in the way that silicon “stores” lithium. A layered graphite structure absorbs lithium ions through a process called intercalation—it essentially consists of sheets of graphene that allow lithium ions to be stored between the layers. Silicon, on the other hand, can absorb more lithium ions, because the two elements form an alloy with a theoretical specific capacity much higher than that of graphite.
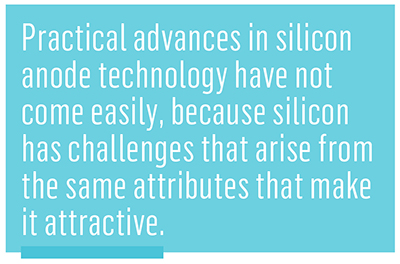
Unfortunately, practical advances in silicon anode technology have not come easily, because silicon has challenges that arise from the same attributes that make it attractive. Unlike porous graphite material, which has specific sites open and waiting for ions, when the lithium-silicon alloy forms, the structure of the anode changes, resulting in large volumetric fluctuations. For example, if a particle of silicon absorbs as much lithium as thermodynamically possible, its volume increases by about 300%. That compares to about 7% expansion observed in the intercalation of lithium into graphite.
The problem with many experimental silicon anodes is that the repeated expansion and contraction during charging and discharging leads to drastically reduced cycle life. So, to use the technology in production batteries, companies have been adding only small amounts of silicon, adding incremental benefits. Basically, they’re still primarily using synthetic graphite, while attempting to increase the amount of silicon in the anode in baby steps over time.
California startup Enevate decided to leapfrog that approach. The company set out to find a way to forego graphite altogether, and build a silicon-dominant anode. Considering that industry heavyweights including LG Chem, Samsung and Renault-Nissan-Mitsubishi have invested $111 million in Enevate to date, the company appears to be onto something.
Enevate recently unveiled its fourth-generation XFC-Energy battery technology (that’s eXtreme Fast Charging), and says it is currently working with multiple automotive OEMs and battery manufacturers to commercialize its technology for 2024-2025 model year EVs.
Charged chatted with Enevate’s Ben Park, founder and CTO, and Jarvis Tou, Executive VP of Marketing and Products, to learn how eliminating graphite from the equation might be the key to unlocking silicon’s potential.
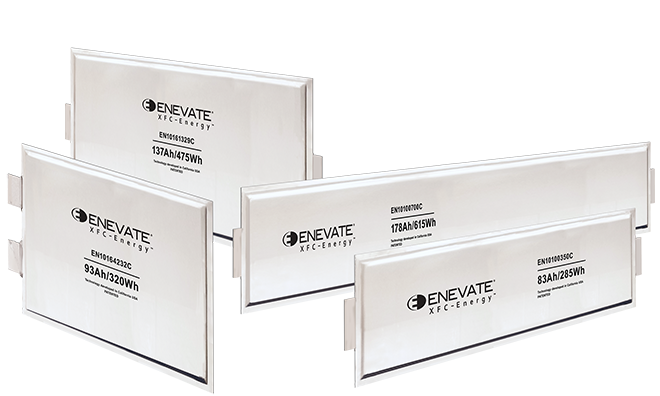
Charged: Could you describe how Enevate’s approach to silicon anode technology is fundamentally different from that of other leaders in the space?
Ben Park: The first thing that’s very important to understand is that others are using evolutionary approaches, and we’re squarely in the non-evolutionary silicon box. For example, the Teslas and the Samsungs of the world are using silicon as an additive to the anode. So, even though they state that they have a silicon anode, it’s basically a graphite anode with a little bit of silicon added to it. There was a huge effort going on for many, many years that increased that percentage level of silicon. And the most common silicon that’s added to these graphite systems is in the form of SiO or SiOx as they call it, basically a silicon oxide.
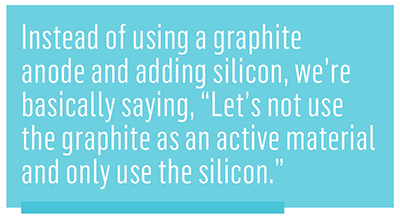
There are a lot of challenges with this approach. First of all, it’s very expensive. Many of the SiO products are over $100 per kilogram. It’s basically an order of magnitude higher than graphite. That’s a problem. Second, it turns out these silicon-based materials have a problem with initial chromic efficiency. So, when you first build a battery, you’ll use up some of the lithium, and you don’t get all of that lithium back. The lithium basically leaks from the cathode, and if you add more, you’ll actually decrease the energy density of the cell. And, of course, the expansion and contraction of silicon is well known, and it causes damage to the electrode, which causes performance issues in cycle life.
Companies are adding the silicon in terms of active material in roughly the 3-8% range, most recently, and struggling to get to 10%.
Our approach is fundamentally different. Instead of using a graphite anode and adding silicon, we’re saying, “Let’s not use the graphite as an active material, and only use the silicon.” As far as we know, we’re the first commercial effort to do this. I think there are now other companies mimicking our approach, but we believe we’re the first.
Charged: Could you explain the technical challenges you overcame to make a functional silicon anode work without graphite?
Ben Park: Within silicon, there are multiple modes damaging the electrode. All of them are tied to expansion and contraction. So, when the silicon absorbs all of the lithium, it expands to roughly 3-4 times its original size. Obviously, that’s an issue. As you charge and discharge the battery, this causes continuous damage to the electrode and the cell. Think of it like blowing up then deflating a balloon. That’s exactly what the silicon is doing.
Now imagine that you’re trying to hold these balloons together. You can try gluing them together with a hard glue, but if you use rubber or plastic to hold them together, it won’t hold very well, because the balloons are continuously expanding and contracting. That’s what people are attempting to do with binders. Originally, people tried to use an extremely flexible binder. If we stick with the balloon analogy, it’s like trying to use a very, very flexible rubber to hold the balloons together. When you expand the balloons, the material between the balloons will expand and contract with the balloons. So far that has not been a success, because unlike a balloon, which will always expand and contract similarly, silicon will expand and contract a little bit differently each time. It’s more like plating and de-plating, like what you see in a lead-acid cell, for example.
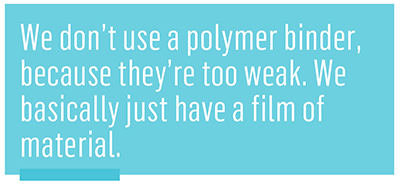
We solved this problem by, first, eliminating the binder. We don’t use a polymer binder, because they’re too weak. We basically just have a film of material. Our approach has been to create an active material piece. It’s basically one piece of powder instead of a billion powder particles held together with a polymer. That way the silicon can expand and contract within an entire rigid structure, and everything is conductive. Even if there is some cracking, it won’t lose its electrical connection to the rest of the material. We went away from polymers because, no matter how rigid or flexible you make a polymer, it doesn’t seem to work.
Our second unique solution has to do with the electrochemistry. If you combine silicon with graphite at all, you’re basically forced to use the silicon “all the way,” so to speak, and that contributes to the cycling problem. Because we don’t use any graphite, we have more options.
Let’s say we have a graphite anode, for example, with lithiation that occurs at around 0.1 volts and lower. What that means is that, as you charge the battery, the anode voltage will be reduced, and then you have to get the voltage to 0.1 volts and lower to have the lithium go into the anode. When you charge the battery, the voltage of the battery increases, but the voltage of the battery is basically the cathode voltage minus the anode voltage. So, as the battery voltage increases, the cathode voltage is increasing and the anode voltage is decreasing. To get the graphite to actually absorb the lithium, you have to get the graphite voltage very low—let’s say below 0.1 V to simplify. Silicon will actually absorb lithium at higher than 0.1 volt—almost all of the lithium will react with silicon at a voltage higher than 0.1 volt.
That means that, if you’re creating a system where you have both graphite and silicon, pretty much all of the silicon reacts first as you’re charging the battery, and then the graphite will react later. So, in a system where you’re trying to get both graphite and silicon to work, you have to utilize the silicon fully. That means that the silicon will expand to 3-4 times its size. It will be very reactive, and will cause problems. In our case, we don’t use that graphite, so we don’t have to bring the silicon to that unstable a level. We don’t have to expand it that much, so we’re able to use silicon in a more reasonable way. We use less of it, and that’s okay because our silicon is less expensive.
By using those two mechanisms [eliminating binders and using less of the available silicon], we were able to solve the major issues with silicon. We also have to make sure our source is readily available and inexpensive, otherwise it would have zero future in the automotive industry. We’re able to use silicon sources that are much cheaper, and much more eco-friendly, meaning a lower CO2 footprint than other companies. And that is key for the automotive industry. We were very happy that our technical approach, which enabled better electrochemical performance, also enabled better price performance and more sustainability.
Charged: How do you control how much of the silicon in a cell is utilized during cycling?
Ben Park: This is just something tied to the design of the battery. All of the lithium in commercial cells today comes in the cathode. Take a high-nickel material like NCA, for example—nickel, cobalt and aluminum. It’s a lithium metal oxide, and all of the lithium comes out of that cathode and goes into the anode, then back and forth. So, what we do, is we design the battery so that the lithium is limited by the cathodes in the battery. So, no matter how much you want to try to charge the battery, you can’t damage the anode.
Charged: If using it fully will expand it 300 to 400%, what would you say the expansion of the silicon in your anodes would be?
Ben Park: On a cell level, we will typically only see maybe 3-8%, which is within the realm of other graphite cells. The silicon itself will still expand 50-100%, depending on cell design. However, it’s actually absorbed within the electrode and the battery design, so you will never see that expansion from the outside.
We don’t have a single cell design, so it’s difficult to say exactly. We choose the cathode depending on the customer. As you know, we’re involved with quite a few automotive efforts now. Each auto company and each battery company have their own preference in terms of cathodes. The good news is, our technology seems compatible with most of the cathodes out there. We have our own reference design, but so far it’s been working well with our partners’ materials and cell designs, along with our help and expertise. Those include the high-nickel cathodes that you may have heard about like nickel-rich NCA, NCM811, NCMA, low-cobalt, or other advanced cathodes.
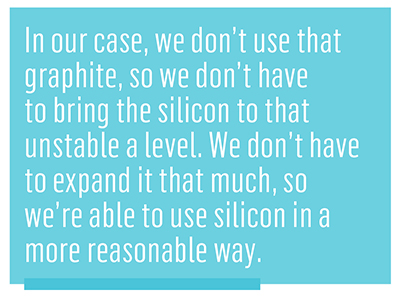
Charged: You’ve said that your new 4th-generation XFC-Energy technology achieves 5-minute charging to 75% capacity with 800 Wh/L cell energy density. What enables such fast charging?
Ben Park: There are multiple factors that contribute to fast charging capabilities. First of all, silicon can react faster, just as a chemical property. Think of a movie theater: if you’re trying to find a seat, it’s going to take a lot longer in a movie theater that’s almost full than in one that’s half-empty. Since we are using silicon without graphite, and we can keep a lot of the silicon empty, it’s a lot easier for the lithium to find a seat.
The second reason is that, because we can keep the voltage higher, it’s much harder to cause lithium plating, which is a huge issue for graphite cells when you’re trying to fast charge. There are other issues as well, but those are the two main reasons.
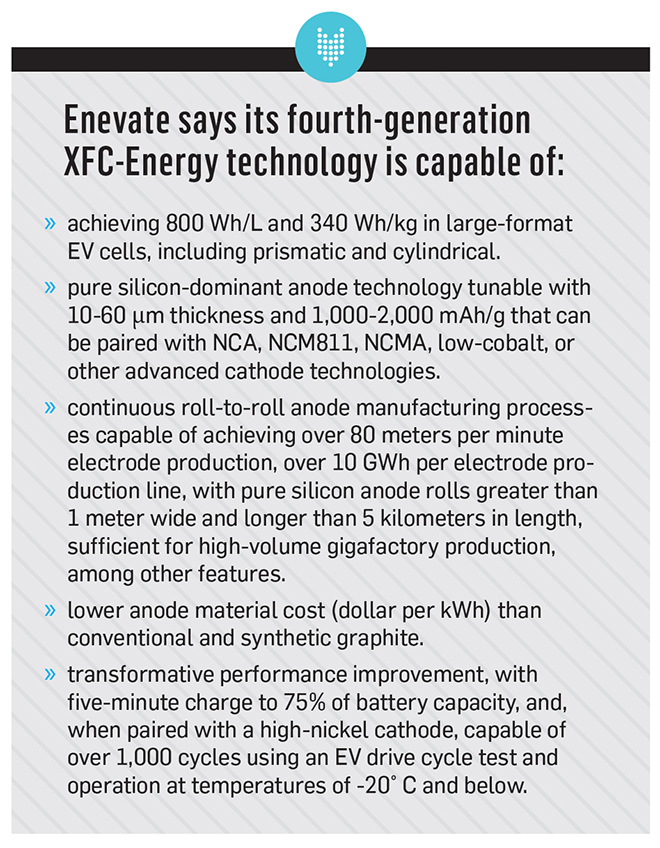
Charged: You’re on your fourth generation of this technology. Have your previous generations been commercialized?
Jarvis Tou: Our first, second, and even third generations were originally designed for consumer electronics. We almost went to market with a cell phone manufacturer to be distributed in the US. It was fully commercialized for volume production, but we had to make a choice as a startup at that point, whether or not to launch into a production model that we would have to sustain for quite some time.
We saw this impending wave coming at us, this massive ramp of EV batteries, just dwarfing consumer electronics and everything else, so we had to ask ourselves: “Do we pivot toward EVs?” That’s ultimately what we did. We ended up not going to market in the consumer electronics space, even though it was fully commercialized. We transitioned to nickel-rich cathodes 3-4 years ago to pursue EV batteries. Our investors directed us to focus on the EV market for the same reasons that you and I are so interested in it today—because there is this massive wave that’s just inevitable, and coming at us and dwarfing everything else.
Ben Park: With a focus on EVs, cycle life was the main thing that we’ve been improving with our technology. Now that we’ve shown a cycle life that’s acceptable to the automakers for the current stage we’re in, we’re focusing on a scale-up. Most of our efforts today are pushing towards scale-up and commercialization.
Our business model is to be a licensing company. We call ourselves a technology provider, because we often work together with the customer to implement the technologies, and we also have a significant manufacturing line where we test and collect information. Most licensing companies just have a patent, but we actually have an operational line. However, we don’t anticipate selling from the line, at least not today. Our main focus is to collect a lot of data so that we can help ramp up the larger-scale production lines, whether it’s battery manufacturers or automotive companies.
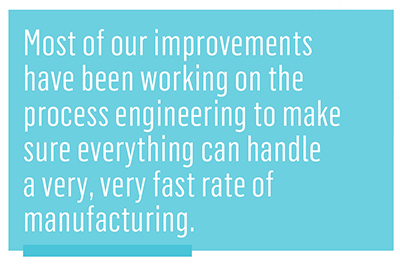
Jarvis Tou: We spend a lot of our time and effort on core R&D. Originally we were targeting to license to battery makers, but we found a shift in the EV industry, as carmakers are realizing the battery is not just a gas tank. It’s actually the powertrain or power plant for the entire EV. As Tesla has demonstrated, it dictates almost everything in an electric car. Weight, performance, handling, price, interior and cargo space—just about everything. And at the powertrain level, many automakers want control. They’re almost taking a page out of Apple’s playbook, which is to own the design of the battery and its supply chain, and license and build the core cell-level and material-level battery technology themselves, and use that as a differentiator. Then they can always contract different cell-makers to make the cells. Or have joint ventures, or even make the cells themselves. That’s what we see going on, and it could be a major shift in the industry.
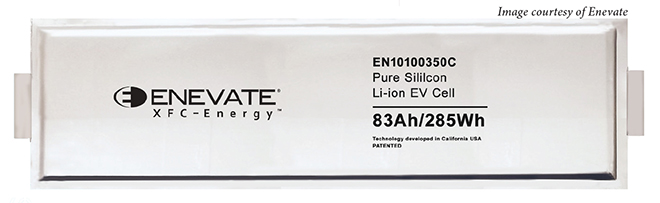
Charged: What were the main attributes that you were focusing on improving while going from third- to fourth-generation XFC-Energy battery technology?
Ben Park: We’ve made some modifications to the cathodes, but most of our improvements have been working on the process engineering to make sure everything can handle a very, very fast rate of manufacturing. For example, everybody we’re working with demands a rate of 80 meters per minute or higher, so we had to work on all the processes to make sure we could handle that.
The fifth generation will be mainly driven by further cost and safety improvements. That’s where solid-state technology may play a role. We don’t know if we actually need solid-state to meet our internal milestones. We’ve set very challenging goals—it’s kind of like the Holy Grail of battery safety that we’re working on for the fifth generation.
Charged: You’ve said that you’re working with OEMs and battery manufacturers to commercialize your technology for 2024-2025 model year EVs. Can you tell me more about the details of that timeline?
Jarvis Tou: New car platforms take 4-6 years to get to production, especially from the traditional automakers. So, we’re on that timeline now—we’re currently designing for the 2024 and 2025 model years.
Ben Park: We are also looking to release the technology in other non-automotive markets earlier. We call them gateway markets. It’s very critical to get the technology out there quickly, and to learn from the technology before it’s released into a very mass-market product like a vehicle. So, our current timetable for that is something like 2 years.
Jarvis Tou: The bottom line is, we can’t take our eye off the ball, which is the EV market. Because of the sheer volume that it presents, and because it plays to our core mission and vision statements, which center around developing innovative battery technologies to accelerate adoption of electrified mobility and help create a cleaner and more sustainable environment for everyone. And that’s why many of our investors and partners believe in us.
This article appeared in Charged Issue 47 – January/February 2020 – Subscribe now.
source https://chargedevs.com/features/enevate-says-its-silicon-dominant-anode-technology-is-ready-for-ev-production/
No comments:
Post a Comment